Mini-Review: SENSORY FEEDBACK IN UPPER EXTREMITY MYOELECTRIC PROSTHESIS
- Elena Hebden
- Dec 1, 2021
- 16 min read
Whilst completing the subject Medical Devices and Diagnostics, we were tasked with creating a mini review article on a subject of our choosing. I decided to write about sensory feedback in prosthetic limbs. I have always been interested in the interaction between the human body and robotics, and this was a perfect task to further my knowledge in a field I am passionate about.
Mini-Review
SENSORY FEEDBACK IN UPPER EXTREMITY MYOELECTRIC PROSTHESIS
Author: Elena Hebden
Affiliation: UTS School of Life Sciences
Correspondence: elena.hebden@student.uts.edu.au
ABSTRACT: Limb loss has a major impact in a patient's life. It creates a disability where the patient can struggle to do daily tasks and interact with their environment, as well as leaving a huge sensory-motor deficit. In this modern day we can develop complex mechatronic systems to advance society and improve quality of life. Amputee victims have many more options in care and are able to have devices that significantly reduce their disability. A number of new devices and surgeries have been developed over the past few decades to reduce phantom limb pain, increase mobility and control of a prosthesis, and ultimately restore arm and hand function. The next frontier in prosthetics is to restore sensations as you would have in a biological hand and arm. Sensory feedback includes tactics (sense of touch), proprioception (awareness of position and movement), temperature and vibration. This paper explores current concepts of myoelectric prosthesis such as Targeted Muscle Reinnervation and Osseointegration for control of a prosthesis, as well as a look into electrode options and the next steps to gain accurate sensory feedback.
1.Introduction
Loss of an upper extremity is an extremely debilitating and life changing event. The physical and psychological implications can negatively impact the patients quality of life (1). While they also find many physical tasks challenging, patients also have a major sensory-motor deficit. This sensory-motor deficit has long been addressed with simple prosthetics. Simple static prosthesis have been around for centuries, as well as body controlled prosthesis using a system of cables and harnesses (1). However this changed after the first and second world wars as there was a large rise of amputee victims. During a time where technology was developing rapidly, this need for better quality prosthetics drove the beginning of Myoelectric prostheses (2).
Myoelectric prosthetics are controlled with the electrical signals generated from muscles, and have been the most recent wave in prosthetic development. Rather than simple mechanical devices, prosthetics are now thought of as complex mechatronic devices.
Today’s myoelectric prostheses generally consist of the physical limb and the mechanical attachment to the body, as well as electrodes to transmit signals across and control the body.
2 . Myoelectric Prostheses
Myoelectric prostheses use electromyographic (EMG) signals from a nearby muscle as a command for the arm to perform certain functions. As the patient contracts their muscle, an electrode reads the electrical activity. For example, antagonistic flexor and extensor muscles are often used to control the opening and closing of the hand (3).
Although surface electrodes have been used historically, they have many limitations. As surface electrodes rely on contact with the skin, they can easily be disrupted by sweating, weight change, or heavy lifting. They can also only collect data from superficial muscles (4).
EMG surface electrode signals are unreliable. Therefore implantable electrodes have become more widely used to transmit these EMG signals. The sensors are implanted directly into the muscle and overcome the limitations of previously used surface electrodes, providing a strong and reliable signal. Pasquina et al. (5) reported that the implanted electrodes provided a smoother and more reliable use.
Using implantable electrodes fixes the reliability of the signal, but not so much the quality. Multichannel arrays can be used to decode the neural code of movement from the EMG signals, rather than just measuring the amplitude. Multichannel electrodes have the ability to estimate the neural drive (4) which can inform the prosthetic device how much force is required for the movement, and can make movements more continuous. Therefore it seems the combination of implanted and multichannel electrodes are the way to go.
Research performed by Muceli et al have combined these techniques to produce an in vivo multi channel electrode that is capable of reading a greater number of motor units (6). Electrode designs will be discussed in greater depth below.
The number of electrodes directly correlates with the degrees of freedom for the prosthesis. Therefore more electrodes are preferred for higher levels of control. However this can be fairly limited and depends on how much residual muscle the patient has left (7). Another option is to use sequential or multistate controllers to cycle through different commands. While this is a solution, it is not user friendly and not intuitive (3).
Target Muscle Reinnervation has been used to overcome this. This method takes a divided nerve from the amputation site and redirects the terminal fibers to a nearby muscle.
A popular example of this is redirecting the nerve to the pectoral muscle. TMR can offer up more control for the device.
3. Targeted Muscle Reinnervation
Targeted muscle reinnervation or TMR, aims to provide greater control of myoelectric prosthesis by creating new EMG signals (8). The surgery involves taking a divided nerve or neuroma, cutting away the damaged parts, combining the divided ends, and redirecting it to a nearby muscle (9). This treatment is not only helpful for controlling myoelectric prostheses; it is also largely applied to help phantom limb and neuroma pain, which many amputee patients suffer from (10).
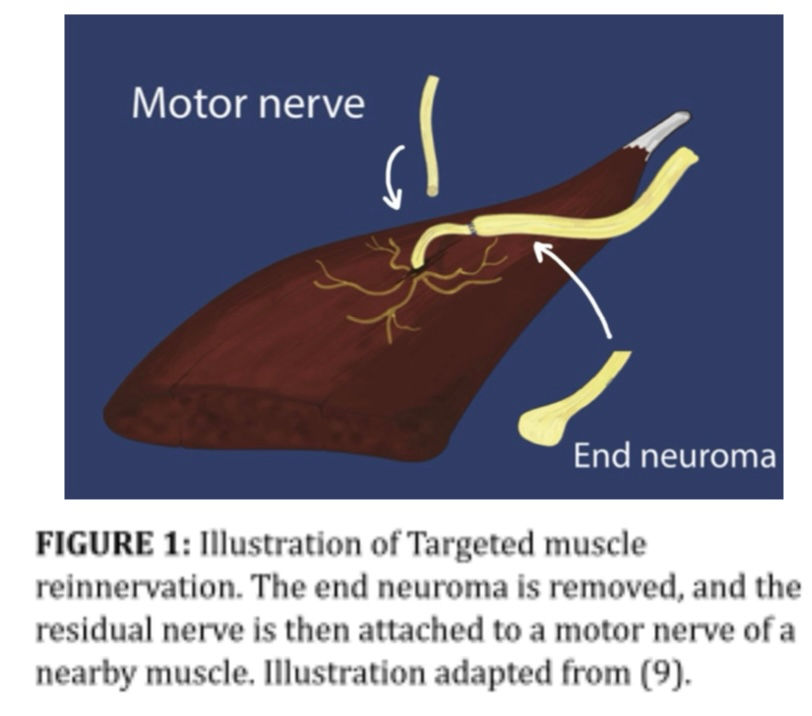
The arm is controlled by the brachial plexus nerve, and after amputation this is still the route of control signals. The terminal nerves on the brachial plexus have slightly different functions, which is favourable for this method as it allows more independent controls (11).
Commonly these nerves are transferred to spare muscles in the chest.. These muscles act as biological amplifiers on the nerve signal, which can be used to control a myoelectric prosthesis (10). TMR also makes it possible for the muscle to receive sensory feedback from the prosthesis (12).
The study completed by Hargrove et al. (7) saw eight males transhumeral amputees that had already undergone TMR compare pattern recognition vs direct control. TMR was a highly successful procedure with Northwestern University reporting an 88.5% success rate of patients operating a TMR-controlled prosthesis. The reinnervated muscles receive more information than the amputation affected nerves which can assist in integrating sensory feedback.
The level or severity of amputation affects TMR as it limits the amount of muscles and nerves available. Patients with unclear nerve injury and severe comorbid injuries are not candidates for TMR (Cheesborough et al., 2014).
4. Osseointegration
The prosthetic arm is historically fixed to the patient with a ‘stump-socket interface’. This design has many drawbacks for patients such as skin irritation, infection, and restricted range of motion (13).
A new technique was developed by a Swedish dentist Per-Ingvar Brånemark in 1969 (14). This method was originally used primarily in dentistry, and has become used more widely in other fields, such as prosthetics, since the 1990s (15). While osseointegration has been available for over 20 years in Europe, the UK and Australia, the FDA has only recently (2015) approved this operation in the United States (16).
Osseointegration is the implanting the medical device directly into the bone. The device consists of two titanium implants; one fixture and one abutment. The medullary cavity is where the titanium fixture is inserted first. The abutment connects the fixture to the prosthesis, protruding outside the body. The abutment and fixture are secured with an abutment screw.
When the titanium is exposed to oxygen inside the body, it forms a titanium O2 layer which then allows the bone to grow into it (17) .
Providing a stable connection between man and machine, osseointegration overcomes the limitations of stump-socket designs, and is more comfortable for the patient. It increases the range of motion and freedom, is fixed more securely and it also allows patients with extreme amputations to have better prosthetic options as the remnant stup does not have to be long (Brånemark, R. et al., 2014). Osseointegration makes it easier to integrate other sensors and devices into the prosthetic due to its compact fit (2) .
Osseointegration can also provide sensory feedback to the user through osseoperception, or mechanoreception through the bone (18). Reliable and accurate sensory feedback is the next frontier in this field, and osseointegration is a route to provide feedback with hardware that is already implanted.
In 2014 a research group from Sweden, including the “father of osseointegration” Brånemark’s son, tested a myoelectric osseointegrated arm with implantable electrodes (19). Their design required bidirectional communication in order to provide sensory feedback to the patient. Feedthrough connectors were cleverly designed to be incorporated into the abutment screw, as well as placed in the tissue on the distal side to link electrodes (as seen in figure 3). The 18 month study reported a stable osseointegrated interface. Although this study had a sample size of 1, therefore more research is required in order to determine the efficacy of this particular technology. It is promising that the implant was able to integrate well with electrodes and provide some sensory feedback. The patient reported sensations such as a “superficial tapping with the tip of a pen,” and commonly “tingling” in the palmar branch of the ulnar nerve of the hand.

Overall osseointegration is a great way to overcome limitations of original prosthetics. While new in the US, it has been used for decades in Europe and proved to last well over time, after many research with high sample sizes such as (18, 20,21)
5. Sensory Feedback
Lack of sensory feedback is a large reason for prosthesis rejection (13). Interestingly many amputees adopt a body controlled prosthesis rather than mainstream myoelectric prosthesis as the harness along the patient's shoulders can give them more sensory feedback (22). Sensory feedback can also reduce phantom limb pain and generally improve the quality of life for amputees (23).
The next stage for prosthetics is receiving accurate and natural sensory feedback to improve patients quality of life and ability to function. The thought behind recreating these signals is that action potentials are electrical signals, so therefore it makes sense that sensations can be recreated with biomechatronics. However it is not as simple as putting together a few sensors into a complete circuit.
In 2005, Dhillion and Horch (23) stimulated amputee nerve stumps to produce sensory feedback. Within individual fascicles of the peripheral nerve, electrodes were implanted to provide discrete sensations of touch and movement. This feedback is used to control the prosthetics movement and grip force by recording motor neuron activity when the patient is attempting to move the phantom limb. This is believed to be the first demonstration of direct neural feedback and control of an artificial arm. The electrodes used are Longitudinal intrafascicular electrodes (LIFEs). LIFEs are designed to be placed inside the peripheral nerve to stimulate as well as record activity. Whilst this electrode was one of the first to record activity, it has since been improved upon, such as changing material to minimise drift and tissue formation around the device, and increase biocompatibility long term (24).
Although a breakthrough of its time, it is important to note that this work is limited with a small sample size and a short trial time. Research did not include data on non-visual, closed-loop control of the arm. However it does produce promising results that are worth investigating further.
A major part of developing high quality sensory feedback is the design and selection of electrodes. Discussed next are popular and innovative electrode designs that have been recently used in research.

6. Different Types of Peripheral Nerve Electrodes
6.1 Cuff Electrode
Naples et al. developed a spiral cuff electrode in 1988 (25). It is designed to wrap itself around the peripheral nerves, which makes the size customizable. The design was tested in a small sample size of 6 cats for 7 months, only slightly affecting intraneural connective tissue.
A research group from the US Veterans affairs (26) displayed a promising study where two upper limb amputees were provided with a natural touch sensation in their “hands” for a prolonged period of 1-2 years. All locations of perceived sensation were consistent with innervation patterns of the nerves in which the cuff electrodes were implanted (median, ulnar and radial nerves). This confirms that the sensory pathways did not re-organise themselves after amputation.
Even when motor and sensory electrodes were on the same nerve, they did not interfere with one another.
Tan et al. were able to produce a “natural” pressure feeling by modulating the intensity of the pulse. Full scale modulation was like a pulse of pressure. One patient described the sensation as a “pulsing pressure, constant pressure, vibration, tapping, and rubbing ona texture”. Small scale, offset modulation felt more like a constant pressure than a pulse. Reducing modulation the pressure became more constant. At minimum modulation the sensation felt like “someone had just laid a finger on my hand”. Overall the study found that intensity modulation could produce a pressure sensation and the intensity of the feeling was controlled by the frequency (26).
6.2 Longitudinal Intrafascicular Electrode (LIFE)
As the name suggests, this electrode is implanted in the nerve longitudinally. Research undertaken in the early 2000s (27) looked into single stranded and multi stranded LIFEs in the sciatic nerve of animals, and compared the two. The polymer based LIFEs were also compared to Pt-Ir based electrodes. As mentioned previously, PPt-Ir wires have demonstrated minimal neural damage and are able to record signals from the nerve, they drift in the nerve which affects the signal to noise ratios and makes them unsuitable for long term use. After this discovery, the polymer based LIFE was developed and is 60 times more flexible than the metal Pt-Ir electrodes. It is manufactured from Kevlar fiber that is 12um in diameter, and is insulated with silicone. The increased flexibility also increases biocompatibility as it can adapt to its surrounding fascicles. This study concluded that the multichannel electrodes were better at recording signals than the single channels; although the signal to noise ratio was lower, which makes it more difficult to distinguish individual signals (selectivity). Therefore the single LIFEs are comparable, but more fragile.
Thin-film longitudinal intrafascicular electrodes tfLIFEs have been developed more recently, and have eight channels per electrode, in comparison to 2 channels per electrode in the original LIFEs (28). However 8 channels is still rather limited for practical use and sensory feedback.
6.3 Transverse Intrafascicular Multichannel Electrode (TIME)
Boretius et al published a highly regarded paper in 2010 that introduced the design of Transverse Intrafascicular Multichannel Electrodes. The transverse implantation of the electrode should allow access to more subgroups of nerve fibers (28). While LIFEs are able to stimulate close nerve fibers to the implantation site (27), the transverse nature of TIME allows it to be capable of interacting with nerve fibers from different fascicles. This means that for the same level of invasiveness as LIFEs, the TIME has a wider range of control.
The original TIME is manufactured from a thick layer of platinum where electrode sites are positioned, which is then sandwiched between polyamide.
More research needs to be conducted into long term use of TIMEs and to determine the best method of fixation to the nerve. For this study by Boreitus et al, the electrode was secured onto the epineurium with surgical thread. The pilot study was performed in rats and experimented with surgical procedure on a human cadaver. Therefore the electrode needed to me altered to fit larger animals and ultimately humans.
More recent studies on TIME electrodes by Kundu et al. (29) demonstrated that Transverse intrafascicular Multichannel Electrodes were capable of higher selectivity (identifying individual signals) that tfLIFEs, as well as accessing a larger number of nerves. Kundu et al. concluded that TIMEs ability to access multiple fascicles with high selectivity will be promising for future myoelectric prostheses.
6.4 USEA
The Utah Slanted Electrode Array (USEA) design was presented by Branner et al in 2000. The USEA is able to access different fibers with 100 multichannel needle electrodes inserted into peripheral nerves with minimal impact into surrounding tissue (30). The slanted design is able to reach more fascicles in the nerve than original Utah Electrode Arrays (UEAs), as the electrodes are different sizes, and reach different parts of the nerve. This design, while a novel idea, needed more research in chronic cases as the author suggests, as well as determining if the invasive procedure will damage the nerve or surrounding tissues in long term human studies, or if large muscle movements will displace the device.
6.5 Regenerative Peripheral Nerve Interfaces (RPNIs)
Regenerative electrodes are a compromise between cuff electrodes, in which their high biocompatibility comes at the price of low selectivity, or penetrating electrodes, whose invasiveness often irritates surrounding tissue in the long term. The concept of regenerative electrodes is to encourage the nerve’s axon to grow toward the electrode (31). Regenerative Peripheral Nerve Interfaces (RPNIs) are constructed from small muscle grafts (1x3cm) from skeletal muscle which is reinnervated with the terminal branches of residual nerves from the amputation site (32). The muscle tissue increases signal amplitude by 50-100 times current peripheral nerve interfaces (31).
While this new method needs more research, a promising study by Frost et al. in 2018 (33) 2) tested RPNIs in rat models for real-time neuroprosthetic control. EMG signals were retrieved successfully and were able to send control signals.
7. Conclusion
Myoelectric prostheses are able to restore extremity function for amputee victims. Treatment surgeries such as osseointegration and targeted muscle reinnervation are currently being used to improve prosthesis control. The next phase for the myoelectric prosthesis is restoring sensory feedback. Osseointegration integrated the device into the bone, which allows the user to receive mechanoreception and also makes it easier to incorporate other devices such as electrodes. Targeted muscle reinnervation redirects neuromas formed after amputation to new muscles that can be used to control the prosthesis.
One of the most important parts of sensory feedback is having a powerful electrode in which to collect and send signals. Over the year many types of electrodes have been developed to collect and transmit signals to and from the nerve such as cuff electrodes, Longitudinal Intrafascicular Electrodes, Transverse Intrafascicular Multichannel Electrodes, USEAs, and Regenerative electrodes. Currently Transverse Intrafascicular Multichannel Electrodes (TIME) show the most promise as they are able to access multiple fascicles with high selectivity, which means they has a wider range of control as well as regenerative electrodes due to their high biocompatibility. Overall, more studies with higher sample sizes are needed in order to advance this field and develop accurate sensory feedback. Osseointegration and targeted muscle reinnervation have proved themselves to work in clinical settings. The next step is developing a powerful electrode that incorporates properties of TIME and RPNIs electrodes.
8. Acknowledgements
Thank you to my tutor William Hansen for giving me great feedback on my draft. Big thank you to my Mum who checked my grammar before submitting.
References
(1) Hernigou P. Ambroise Paré IV: The early history of artificial limbs (from robotic to prostheses). International orthopaedics 2013;37(6):1195-1197.
(2) Bates TJ, Fergason JR, Pierrie SN. Technological Advances in Prosthesis Design and Rehabilitation Following Upper Extremity Limb Loss. Current reviews in musculoskeletal medicine 2020 Aug;13(4):485-493.
(3) Aman M, Festin C, Sporer ME, Gstoettner C, Prahm C, Bergmeister KD, et al. Bionic reconstruction : Restoration of extremity function with osseointegrated and mind-controlled prostheses. Wiener Klinische Wochenschrift 2019 Dec;131(23-24):599-607.
(4) Aman M, Sporer ME, Gstoettner C, Prahm C, Hofer C, Mayr W, et al. Bionic hand as artificial organ: Current status and future perspectives. Artificial Organs 2019 Feb;43(2):109-118.
(5) Pasquina PF, Evangelista M, Carvalho AJ, Lockhart J, Griffin S, Nanos G, et al. First-in-man demonstration of a fully implanted myoelectric sensors system to control an advanced electromechanical prosthetic hand. Journal of neuroscience methods 2015;244:85-93.
(6) Muceli S, Poppendieck W, Negro F, Yoshida K, Hoffmann KP, Butler JE, et al. Accurate and representative decoding of the neural drive to muscles in humans with multi‐channel intramuscular thin‐film electrodes. The Journal of Physiology 2015 Sep 1,;593(17):3789-3804.
(7) Hargrove LJ, Miller LA, Turner K, Kuiken TA. Myoelectric Pattern Recognition Outperforms Direct Control for Transhumeral Amputees with Targeted Muscle Reinnervation: A Randomized Clinical Trial. Scientific reports 2017;7(1):13840-9.
(8) Myers H, Lu D, Gray SJ, Bruscino‐Raiola F. Targeted muscle reinnervation to improve electromyography signals for advanced myoelectric prosthetic limbs: a series of seven patients. ANZ J Surg 2020;90(4):591-596.
(9) Mioton LM, Dumanian GA. Targeted muscle reinnervation and prosthetic rehabilitation after limb loss. Journal of surgical oncology 2018;118(5):807-814.
(10) Cheesborough JE, Smith LH, Kuiken TA, Dumanian GA. Targeted Muscle Reinnervation and Advanced Prosthetic Arms. Seminars in Plastic Surgery 2015 Feb;29(1):62.
(11) Kuiken TA, Dumanian GA, Lipschutz RD, Miller LA, Stubblefield KA. The use of targeted muscle reinnervation for improved myoelectric prosthesis control in a bilateral shoulder disarticulation amputee. Prosthet Orthot Int 2004;28(3):245-253.
(12) Kuiken TA, Li G, Lock BA, Lipschutz RD, Miller LA, Stubblefield KA, et al. Targeted Muscle Reinnervation for Real-time Myoelectric Control of Multifunction Artificial Arms. JAMA : the journal of the American Medical Association 2009 Feb 11,;301(6):619-628.
(13) Biddiss EA, Chau TT. Upper limb prosthesis use and abandonment: A survey of the last 25 years. Prosthetics and Orthotics International 2016;31(3):236-257.
(14) Brånemark P-, Breine U, Adell R, Hansson BO, Lindström J, Ohlsson Å. Intra-Osseous Anchorage of Dental Prostheses: I. Experimental Studies. Scandinavian journal of plastic and reconstructive surgery 2009;3(2):81-100.
(15) Li Y, Brånemark R. Osseointegrated prostheses for rehabilitation following amputation. Der Unfallchirurg 2017;120(4):285-292.
(16) Al Muderis M, Lu W, Tetsworth K, Bosley B, Li JJ. Single-stage osseointegrated reconstruction and rehabilitation of lower limb amputees: the Osseointegration Group of Australia Accelerated Protocol-2 (OGAAP-2) for a prospective cohort study. BMJ Open 2017 Mar;7(3):e013508.
(17) Jayesh RS, Dhinakarsamy V. Osseointegration. Journal of pharmacy & bioallied science 2015 Apr;7(Suppl 1):S226-S229.
(18) Jönsson S, Caine-Winterberger K, Brånemark R. Osseointegration amputation prostheses on the upper limbs: methods, prosthetics and rehabilitation. Prosthetics and orthotics international 2011;35(2):190-200.
(19) Ortiz-Catalan M, Hakansson B, Branemark R. An osseointegrated human-machine gateway for long-term sensory feedback and motor control of artificial limbs. Science translational medicine 2014;6(257):257re6.
(20) Brånemark R, Berlin O, Hagberg K, Bergh P, Gunterberg B, Rydevik B. A novel osseointegrated percutaneous prosthetic system for the treatment of patients with transfemoral amputation: A prospective study of 51 patients. The bone & joint journal 2014 Jan;96-B(1):106-113.
(21) Hagberg K, Brånemark R, Hagberg K. One hundred patients treated with osseointegrated transfemoral amputation prostheses--rehabilitation perspective. Journal of rehabilitation research and development 2009;46(3):331-344.
(22) Antfolk C, D’Alonzo M, Rosén B, Lundborg G, Sebelius F, Cipriani C. Sensory feedback in upper limb prosthetics. Expert review of medical devices 2014;10(1):45-54.
(23) Dhillon GS, Horch KW. Direct Neural Sensory Feedback and Control of a Prosthetic Arm. TNSRE 2005;13(4):468-472.
(24) Lawrence SM, Dhillon GS, Horch KW. Fabrication and characteristics of an implantable, polymer-based, intrafascicular electrode. Journal of neuroscience methods 2003;131(1-2):9-26.
(25) Naples GG, Mortimer JT, Scheiner A, Sweeney JD. A spiral nerve cuff electrode for peripheral nerve stimulation. TBME 1988 Nov;35(11):905-916.
(26) Tan DW, Schiefer MA, Keith MW, Anderson JR, Tyler J, Tyler DJ. A neural interface provides long-term stable natural touch perception. Science translational medicine 2014;6(257):257ra138.
(27) Lawrence SM, Dhillon GS, Jensen W, Yoshida K, Horch KW. Acute peripheral nerve recording Characteristics of polymer-based longitudinal intrafascicular electrodes. TNSRE 2004 Sep;12(3):345-348.
(28) Boretius T, Badia J, Pascual-Font A, Schuettler M, Navarro X, Yoshida K, et al. A transverse intrafascicular multichannel electrode (TIME) to interface with the peripheral nerve. Biosensors & bioelectronics 2010;26(1):62-69.
(29) Kundu A, Harreby KR, Yoshida K, Boretius T, Stieglitz T, Jensen W. Stimulation Selectivity of the "Thin-Film Longitudinal Intrafascicular Electrode" (tfLIFE) and the "Transverse Intrafascicular Multi-Channel Electrode" (TIME) in the Large Nerve Animal Model. TNSRE 2014 Mar;22(2):400-410.
(30) Almut Branner, Richard B. Stein, Richard A. Normann. Selective Stimulation of Cat Sciatic Nerve Using an Array of Varying-Length Microelectrodes. Journal of Neurophysiology 2001 Apr 1,;85(4):1585-1594.
(31) Thompson CH, Zoratti MJ, Langhals NB, Purcell EK. Regenerative Electrode Interfaces for Neural Prostheses. Tissue engineering. Part B, Reviews 2016;22(2):125-135.
(32) Frost CM, Ursu DC, Flattery SM, Nedic A, Hassett CA, Moon JD, et al. Regenerative peripheral nerve interfaces for real-time, proportional control of a Neuroprosthetic hand. Journal of neuroengineering and rehabilitation 2018;15(1):108.
(33) Frost CM, Ursu DC, Flattery SM, Nedic A, Hassett CA, Moon JD, et al. Regenerative peripheral nerve interfaces for real-time, proportional control of a Neuroprosthetic hand. Journal of neuroengineering and rehabilitation 2018;15(1):108.
(34) Jönsson S, Caine-Winterberger K, Brånemark R. Osseointegration amputation prostheses on the upper limbs: methods, prosthetics and rehabilitation. Prosthetics and orthotics international 2011;35(2):190-200.
(35) Frolke JPM, Leijendekkers RA, Meent Hvd. Osseointegrated prosthesis for patients with an amputation : Multidisciplinary team approach in the Netherlands. Unfallchirurg 2017;120(4):293-299.
(36) Albrektsson T, Albrektsson B. Osseointegration of bone implants: A review of an alternative mode of fixation. Acta orthopaedica Scandinavica 2009;58(5):567-577.
(37) Al Muderis M, Lu W, Tetsworth K, Bosley B, Li JJ. Single-stage osseointegrated reconstruction and rehabilitation of lower limb amputees: the Osseointegration Group of Australia Accelerated Protocol-2 (OGAAP-2) for a prospective cohort study. BMJ Open 2017 Mar;7(3):e013508.
(38) Rijnbeek EH, Eleveld N, Olthuis W. Update on Peripheral Nerve Electrodes for Closed-Loop Neuroprosthetics. Frontiers in neuroscience 2018;12:350.
(39) Oddo CM, Raspopovic S, Artoni F, Mazzoni A, Spigler G, Petrini F, et al. Intraneural stimulation elicits discrimination of textural features by artificial fingertip in intact and amputee humans. eLife 2016 Mar 8,;5:e09148.
(40) Malagodi MS, Horch KW, Schoenberg AA. An intrafascicular electrode for recording of action potentials in peripheral nerves. Annals of biomedical engineering 1989;17(4):397-410.
(41) Tyler D, Durand D. Chronic Response of the Rat Sciatic Nerve to the Flat Interface Nerve Electrode. Annals of Biomedical Engineering 2003 Jun;31(6):633-642.
(42) Pierrie SN, Gaston RG, Loeffler BJ. Current Concepts in Upper-Extremity Amputation. Journal of Hand Surgery 2018 Jul;43(7):657-667.
(43) Hruby LA, Sturma A, Mayer JA, Pittermann A, Salminger S, Aszmann OC. Algorithm for bionic hand reconstruction in patients with global brachial plexopathies. Journal of neurosurgery 2017 Nov;127(5):1163-1171.
(44) Rossini PM, Micera S, Benvenuto A, Carpaneto J, Cavallo G, Citi L, et al. Double nerve intraneural interface implant on a human amputee for robotic hand control. Clinical Neurophysiology 2010;121(5):777-783.
(45) Yildiz KA, Shin AY, Kaufman KR. Interfaces with the peripheral nervous system for the control of a neuroprosthetic limb: a review. Journal of neuroengineering and rehabilitation 2020 Mar 10,;17(1):1-43.
(46) Bergmeister K, Hader M, Lewis S, Russold M, Schiestl M, Manzano-Szalai K, et al. Prosthesis Control with an Implantable Multichannel Wireless Electromyography System for High-Level Amputees: A Large-Animal Study. Plastic and reconstructive surgery (1963) 2016 Jan;137(1):153-162.
Comments